Greenhouse gas emissions and carbon sequestration by agroforestry systems in southeastern Brazil
Agrosilvopastoral and silvopastoral systems can increase carbon sequestration, offset greenhouse gas (GHG) emissions and reduce the carbon footprint generated by animal production. The objective of this study was to estimate GHG emissions, the tree and grass aboveground biomass production and carbon storage in different agrosilvopastoral and silvopastoral systems in southeastern Brazil. The number of trees required to offset these emissions were also estimated. The GHG emissions were calculated based on pre-farm (e.g. agrochemical production, storage, and transportation), and on-farm activities (e.g. fertilization and machinery operation). Aboveground tree grass biomass and carbon storage in all systems was estimated with allometric equations. GHG emissions from the agroforestry systems ranged from 2.81 to 7.98 t CO2e ha −1 . Carbon storage in the aboveground trees and grass biomass were 54.6, 11.4, 25.7 and 5.9 t C ha −1 , and 3.3, 3.6, 3.8 and 3.3 t C ha −1 for systems 1, 2, 3 and 4, respectively. The number of trees necessary to offset the emissions ranged from 17 to 44 trees ha −1 , which was lower than the total planted in the systems. Agroforestry systems sequester CO2 from the atmosphere and can help the GHG emission-reduction policy of the Brazilian government.
Similar content being viewed by others
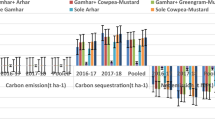
Carbon sequestration and credit potential of gamhar (Gmelina arborea Roxb.) based agroforestry system for zero carbon emission of India
Article Open access 28 February 2024
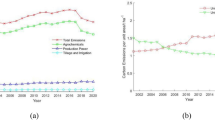
Inter-annual variation patterns in the carbon footprint of farmland ecosystems in Guangdong Province, China
Article Open access 19 August 2022
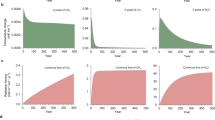
Risk to rely on soil carbon sequestration to offset global ruminant emissions
Article Open access 22 November 2023
Introduction
The Paris Agreement, adopted in the 21st session of the Conference of the Parties (COP 21) for the United Nations Framework Convention on Climate Change (UNFCCC), aims to maintain the global average temperature below 2 °C of pre-industrial levels 1 . The signatory countries stipulate their Intended Nationally Determined Contributions (INDCs), which are the main commitments and contributions of that country for the fulfillment of the agreement 2,3 .
The Brazilian INDC proposed to reduce the greenhouse gases (GHG) emission by 37% in 2025, based on 2005 levels 4 . Agriculture is the main emission source with enteric fermentation being responsible for 90% of CH4 and animal manure on pasture for 33% of N2O emissions in Brazil in 2014 5 . The Brazilian government established a “low-carbon agriculture plan” to promote sustainable practices in agriculture by reducing greenhouse gas (GHG) emissions while maintaining profitability 6 .
This plan is based on practices such as restoration of degraded pastures, crop-livestock-forest integration, no-till farming, biological nitrogen fixation and forestry and agroforestry systems 6 . The agroforestry system is a land use management system combining trees and/or woody perennial plants, pasture and livestock benefiting from ecological and economic interactions between its component parts due to production diversification 7 . Food production 8 and carbon sequestration by tree planting 9 in these systems can help to reduce deforestation in tropical countries 10,11 .
Agrosilvopastoral and silvopastoral systems are agroforestry system types that can reduce and offset GHG emissions from the Brazilian agricultural sector, mainly using cattle and forest integration 12,13,14 . These systems lower animal emission levels 12 by improving grass quality, which can reduce CH4 emissions from enteric fermentation 15 and digestion efficiency 16 . Furthermore, these systems may mitigate GHG emissions by enhancing carbon sequestration through increasing above and belowground biomass 17,18,19 .
The objective of this study was to estimate GHG emissions, tree and grass aboveground biomass and carbon storage in silvopastoral and agrosilvopastoral systems in southeastern Brazil, and the number of trees required to offset these emissions.
Results
GHG emissions
The pre-farm GHG emissions were 0.37, 0.15, 0.12 and 0.10 t CO2e ha-1 in systems 1, 2, 3 and 4, respectively. Nitrogen production was the main emission source for pre-farm activities (Fig. 1). On-farm GHG emissions were 7.61, 4.10, 3.92 and 2.71 t CO2e ha −1 in systems 1, 2, 3 and 4, respectively. Enteric fermentation and manure produced by livestock were the main emission sources for on-farm activity (Fig. 1). Total GHG emissions were 7.98, 4.25, 4.04 and 2.80 t CO2e ha −1 , on systems 1, 2, 3 and 4, respectively (Table 1).
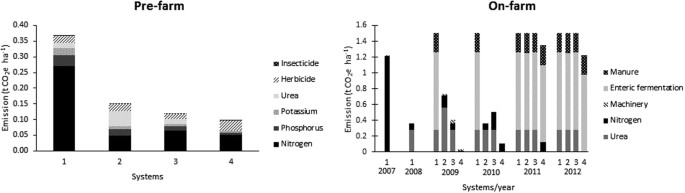

Pre-farm emissions were calculated using emission factors (Table 5) 51 and the following equation: emAgr = agrochemical *EF*(44/12), EmAgr = annual emissions resulting from production, packaging, storage and distribution of agrochemicals, kg CO2 year −1 ; agrochemical = agrochemical applied, kg year −1 ; EF = emission factor, kg carbon equivalent kg −1 ; 44/12 = C to CO2 conversion factor.
Table 5 Carbon emissions (mean ± SD) for the production, transportation, storage, and transfer of agrochemicals. Values according to a previous study 51 .
On-farm emissions were calculated based on the “Guidelines for National Greenhouse Gas Inventories” 52 . GHG sources included nitrogen fertilization, farm machinery, enteric fermentation, and manure management.
Input emissions from synthetic fertilizers were calculated via two pathways: direct and indirect. The direct emissions refer to mineral fertilizer applications 52 . Direct emissions are the product of the nitrogen applied by the emission factor (0.01) 52 using the 44/28 factor to convert N2 to N2O, and N2O global warming potential (298 units of CO2e) 53 . The equation used to estimate direct emissions was: EmDiF = FSN/FRP*EF1*(44/28)*GWP; EmDiF = direct CO2e emissions from N inputs to managed soils, kg CO2 ha −1 ; FSN = annual amount of synthetic fertilizer N applied to soils, kg N ha −1 ; FPRP = annual amount of dung and urine N deposited on soils, kg N −1 ; EF1 = emission factor developed for N2O emissions from synthetic fertilizer, kg N2O–N (kg N) −1 ; 44/28 = N2 to N2O conversion factor; GWP = global warming potential.
Indirect emissions result from volatilization, atmospheric deposition of NH3 and NOx, and nitrogen leaching and runoff from the fertilizers 54,55 . Indirect emissions were calculated using annual amount of fertilizer N applied to soils and the nitrogen fraction lost by volatilization, leaching and/or runoff 56 . The emission factor was 0.01 for volatilization and 0.0075 for leaching/runoff. The nitrogen fraction lost due to volatilization and leaching/runoff was fixed as 0.1 and 0.2, respectively 52 . The equation used to estimate indirect on-farm N2O emissions per system was EmLnL = FSN*FracLEACH-(H)*EF3*(44/28)*GWP, where EmLnL = amount of CO2e produced from additions to managed soils, kg CO2 ha −1 ; FSN = amount of synthetic fertilizer N applied to soils, kg N ha −1 ; EF3 = emission factor for N2O emissions from N leaching and runoff, kg N2O–N (kg N leached and runoff) −1 ; FracLEACH-(H) = fraction of all N added to/mineralized in managed soils in regions where leaching/runoff occurs that is lost through leaching and runoff, kg N (kg of N additions) −1 ; GWP = global warming potential.
NO2 emissions from urea were calculated with the same equations used for the other nitrogen fertilizers. CO2 emissions were the product of the urea applied to the soil by its emission factor, 0.20 52 . The equation used to estimate on-farm CO2 emissions was EmUrea = M*EF4, where EmUrea = amount of CO2e produced from urea application, t CO2 ha −1 ; M = amount of urea applied to soils, t N ha −1 ; EF4 = emission factor for applied urea, t of C (ton of urea) −1 .
CO2 emissions from agricultural machinery were those generated by fuel consumption during eucalypt planting due to its emission factor (EF5), 2.327 kg CO2 −1 52 . The equation used in each system was EmD = F*EF5, where EmD = amount of CO2e produced from fuel consumed, kg CO2 ha −1 ; F = fuel consumed, L ha −1 ; EF5 = emission factor, kg C (L fuel) −1 .
The CH4 emissions by enteric fermentation from cattle were calculated using the factor of 39 kg CH4 year −1 animal unit −1 57 . The equation used was: EmFE = N* EF6* GWP, where EmFE = emissions from enteric fermentation, kg CO2 ha −1 ; N = number of animals, head ha −1 ; EF6 = emission factor for enteric fermentation (kg CH4) head −1 ; GWP = CH4 global warming potential. N2O emissions due to manure deposition were calculated with the same equations as those for nitrogen fertilizer.
Carbon storage in aboveground biomass
Ten pasture grass samples (1 m 2 ) between tree rows were collected, per season, from June 2012 to October 2013. Their fresh weight was obtained and the fresh:dry weight ratio calculated with 25 g from each sample. These samples were dried at approximately 65 °C in an oven until weight stabilization.
The diameter at breast height (DBH), total height, and commercial height (stem height up to 3-cm diameter) of trees per system were measured between July and August 2012. Trees were grouped into DBH classes, and three individuals per class were selected and felled to determine their total volume, biomass and carbon levels in their stem, branches and leaves.
The trees selected were cut at ground level, and the stem diameters measured at 0.3, 0.7, and 1.3 m from their base, and thereafter at every 2 m until the diameter reached 3 cm. The volume of these stem sections was calculated using the Smalian’s formula 58 . The stems per sample were weighed and 2.5 cm thick stem discs were collected at the base, 25, 50, 75, and 100% of the commercial height to calculate the aboveground biomass. An additional stem disc was cut at breast height (1.3 m). The branches and stem discs were dried at 103 ± 2 °C until dry weight stabilization was reached. The leaf and branch weights per tree sampled were recorded. Fresh leaf and branch samples were weighed in the field, stored in bags and sent to the laboratory to determine their dry/fresh weight ratio 59 . Leaf and branch samples were dried at 65 ± 2 °C until dry weight stabilization.
The stem, leaf and branch carbon content was determined with a LECO TruSpec Micro CHN analyzer (LECO Corp., St. Joseph, MI). The carbon stock was obtained by multiplying the aboveground biomass by the carbon content.
Field data was fitted to allometric equations 60,61 to estimate the tree aboveground biomass, and carbon (stem + branches + leaves) per system as: Y1 = β01*DBH β11 *H β21 *ε1; Y2 = β02*(DBH 2 *H) β12 *ε2, whereYj the biomass or carbon stock (kg) of the j th model; H total height (m); β0i, β1i, and β2i the parameters of the j th model and εi:the random errors.
All statistical analyses were performed with R statistical software 62 . The best equations were based on the criteria: parameter significance (p < 0.05) by Wald test; coherence of the sign associated with a specific parameter; goodness of fit statistics: R 2 adj = 1 − [(n − p − 1)/(n − p)] * (1 − R 2 ); R 2 = 1 − [Σ(y − \(\hat\) ) 2 /Σ(y − \(\bar\) ) 2 ); RMES% = (100/ \(\overline\) ) * \(\sqrt>(y-\hat)2/n>\) ; \(\bar \% \) = (100/ \(\bar\) ) * (Σ(y − \(\hat\) )/n), where, R 2 is the empirical determination coefficient or model efficiency; R 2 adj , an empirical adjusted determination coefficient; \(\bar\) %, a relative bias; RMSE%, the root square error in percentage; n, the observation number; p, the number of explanatory variables; \(\bar\) , the mean of dependent variable (volume, biomass and carbon); yi, the i th observed value; and \(\hat\) , the i th value of the dependent variable.
References
- UNFCCC. Adoption of the Paris Agreement. (2015).
- Rose, A., Wei, D., Miller, N. & Vandyck, T. Equity, Emissions Allowance Trading and the Paris Agreement on Climate Change. Econ. Disasters Clim. Change1, 203–232 (2017). ArticleGoogle Scholar
- Rogelj, J. et al. Paris Agreement climate proposals need a boost to keep warming well below 2 °C. Nature534, 631–639 (2016). ArticleADSCASPubMedGoogle Scholar
- Brazil. Intended nationally determined contribution towards achieving the objective of the United Nations Framework Convention on climate change. (2015).
- MCTI. Estimativas anuais de emissões de gases de efeito estufa. (2016).
- Brazil. Plano Setorial de Mitigação e de Adaptação às Mudanças Climáticas para a Consolidação de uma Economia de Baixa Emissão de Carbono na Agricultura. (2011).
- Nair, P. K. R. An introduction to agroforestry. (Kluwer Academic Publishers, 1993).
- Reed, J. et al. Trees for life: The ecosystem service contribution of trees to food production and livelihoods in the tropics. For. Policy Econ.84, 62–71 (2017). ArticleGoogle Scholar
- Zomer, R. J. et al. Global Tree Cover and Biomass Carbon on Agricultural Land: The contribution of agroforestry to global and national carbon budgets. Sci. Rep.6, 29987 (2016). ArticleADSCASPubMedPubMed CentralGoogle Scholar
- Oelbermann, M., Paul Voroney, R. & Gordon, A. M. Carbon sequestration in tropical and temperate agroforestry systems: a review with examples from Costa Rica and southern Canada. Agric. Ecosyst. Environ.104, 359–377 (2004). ArticleCASGoogle Scholar
- Ibrahim, M., Guerra, L., Casasola, F. & Neely, C. Importance of silvopastoral systems for mitigation of climate change and harnessing of environmental benefits. in Grassland carbon sequestration: Management, policy and economics. Proceedings of the workshop on the role of grassland carbon sequestration in the mitigation of climate change. (eds Abberton, M., Conant, R. & Batello, C.) 189–196 (Food and Agriculture Organization of the United Nations, 2010).
- Nair, P. K. R., Tonucci, R. G., Garcia, R. & Nair, V. D. Silvopasture and carbon sequestration with special reference to the Brazilian Savanna (Cerrado). in Carbon sequestration potential of agroforestry systems (eds Kumar, B. M. & Nair, P. K. R.) 145–162 (Springer Netherlands, 2011).
- Balbino, L. C., Cordeiro, L. A. M. & Martínez, G. B. Contributions of the crop-livestock-forest integration systems (iLPF) for a low carbon emission agriculture. Rev. Bras. Geogr. Física4, 1163–1175 (2012). Google Scholar
- Peters, M. et al. Tropical Forage-based Systems to Mitigate Greenhouse Gas Emissions. in Eco-Efficiency:From Visionto Reality (eds. Hershey, C. H. & Paul Neate) 171–190 (Centro Internacional de Agricultura Tropical (CIAT), 2013).
- Bernardi, R. E., de Jonge, I. K. & Holmgren, M. Trees improve forage quality and abundance in South American subtropical grasslands. Agric. Ecosyst. Environ.232, 227–231 (2016). ArticleGoogle Scholar
- Thornton, P. K. & Herrero, M. Potential for reduced methane and carbon dioxide emissions from livestock and pasture management in the tropics. Proc. Natl. Acad. Sci. USA107, 19667–19672 (2010). ArticleADSCASPubMedPubMed CentralGoogle Scholar
- Dube, F. et al. Productivity and carbon storage in silvopastoral systems with Pinus ponderosa and Trifolium spp., plantations and pasture on an Andisol in Patagonia, Chile. Agrofor. Syst.86, 113–128 (2012). ArticleGoogle Scholar
- Paula, R. R. et al. Eucalypt growth in monoculture and silvopastoral systems with varied tree initial densities and spatial arrangements. Agrofor. Syst.87, 1295–1307 (2013). ArticleGoogle Scholar
- Nair, P. K. R. Climate change mitigation: A low-hanging fruit of agroforestry. in Agroforestry - The future of global landUse (eds Nair, P. K. R. & Garrity, D.) 31–67 (Springer Netherlands, 2012).
- Browne, N. A., Eckard, R. J., Behrendt, R. & Kingwell, R. S. A comparative analysis of on-farm greenhouse gas emissions from agricultural enterprises in south eastern Australia. Anim. Feed Sci. Technol.166–167, 641–652 (2011). ArticleGoogle Scholar
- Pradhan, P., Reusser, D. E. & Kropp, J. P. Embodied Greenhouse Gas Emissions in Diets. Plos One8, e62228 (2013). ArticleADSCASPubMedPubMed CentralGoogle Scholar
- Stavi, I. & Lal, R. Agroforestry and biochar to offset climate change: a review. Agron. Sustain. Dev.33, 81–96 (2013). ArticleGoogle Scholar
- West, T. O. & Post, W. M. Soil organic carbon sequestration rates by tillage and crop rotation. Soil Sci. Soc. Am. J.66, 1930–1946 (2002). ArticleADSCASGoogle Scholar
- Six, J. et al. The potential to mitigate global warming with no-tillage management is only realized when practised in the long term. Glob. Change Biol.10, 155–160 (2004). ArticleADSGoogle Scholar
- Thomas, G. A., Dalal, R. C. & Standley, J. No-till effects on organic matter, pH, cation exchange capacity and nutrient distribution in a Luvisol in the semi-arid subtropics. Soil Tillage Res.94, 295–304 (2007). ArticleGoogle Scholar
- Varvel, G. E. & Wilhelm, W. W. No-tillage increases soil profile carbon and nitrogen under long-term rainfed cropping systems. Soil Tillage Res.114, 28–36 (2011). ArticleGoogle Scholar
- Killham, K. Integrated soil management – moving towards globally sustainable agriculture. J. Agric. Sci.149, 29–36 (2011). ArticleGoogle Scholar
- Souza, de. et al. Protective shade, tree diversity and soil properties in coffee agroforestry systems in the Atlantic Rainforest biome. Agric. Ecosyst. Environ.146, 179–196 (2012). ArticleGoogle Scholar
- Delgado, M. E. M. & Canters, F. Modeling the impacts of agroforestry systems on the spatial patterns of soil erosion risk in three catchments of Claveria, the Philippines. Agrofor. Syst.85, 411–423 (2012). ArticleGoogle Scholar
- Norwood, C. A. Water use and yield of limited-irrigated and dryland corn. Soil Sci. Soc. Am. J.64, 365–370 (2000). ArticleADSCASGoogle Scholar
- Laclau, J.-P. et al. Mixing Eucalyptus and Acacia trees leads to fine root over-yielding and vertical segregation between species. Oecologia172, 903–913 (2013). ArticleADSPubMedGoogle Scholar
- Albrecht, A. & Kandji, S. T. Carbon sequestration in tropical agroforestry systems. Agric. Ecosyst. Environ.99, 15–27 (2003). ArticleCASGoogle Scholar
- Jose, S. Agroforestry for ecosystem services and environmental benefits: an overview. Agrofor. Syst.76, 1–10 (2009). ArticleGoogle Scholar
- Mosquera-Losada, M. R., Freese, D. & Rigueiro-Rodríguez, A. Carbon sequestration in european agroforestry systems. in Carbon sequestration potential of agroforestry systems 43–59 (Springer, Dordrecht, 2011).
- Leite, H. G., Nogueira, G. S. & Moreira, A. M. Effect of spacing and age on stand variables of Pinus Taeda L. Rev. Árvore30, 603–612 (2006). ArticleGoogle Scholar
- Bernardo, A. L., Reis, M. G. F., Reis, G. G., Harrison, R. B. & Firme, D. J. Effect of spacing on growth and biomass distribution in Eucalyptus camaldulensis, E. pellita and E. urophylla plantations in southeastern Brazil. For. Ecol. Manag.104, 1–13 (1998). ArticleGoogle Scholar
- Harrington, T. B., Harrington, C. A. & DeBell, D. S. Effects of planting spacing and site quality on 25-year growth and mortality relationships of Douglas-fir (Pseudotsuga menziesii var. menziesii). For. Ecol. Manag.258, 18–25 (2009). ArticleGoogle Scholar
- Oliveira Neto, S. N. et al. Sistema agrossilvipastoril: Integração lavoura, pecuária e floresta. (Sociedade de Investigações Florestais, 2010).
- Blanco-Canqui, H. & Lal, R. Agroforestry. in Principles of soil conservation and management 259–283 (Springer Netherlands, 2010).
- Silva, G. L., Lima, H. V., Campanha, M. M., Gilkes, R. J. & Oliveira, T. S. Soil physical quality of Luvisols under agroforestry, natural vegetation and conventional crop management systems in the Brazilian semi-arid region. Geoderma167–168, 61–70 (2011). ArticleGoogle Scholar
- Müller, M. D., Fernandes, E. N., de Castro, C. R. T., Paciullo, D. S. C. & de Alves, F. F. Estimativa de acúmulo de biomassa e carbono em sistema agrossilvipastoril na Zona da Mata Mineira. Pesqui. Florest. Bras.60, 11–17 (2009). Google Scholar
- Tsukamoto Filho, A. et al. L. da. Fixação de carbono em um sistema agrissilvipastoril com eucalipto na região do cerrado de Minas Gerais. Rev. Agrossilvicultura1, 29–41 (2004). Google Scholar
- Gutmanis, D. Estoque de carbono e dinâmica ecofisiológica em Sistemas Silvipastoris. (Universidade Estadual Paulista, 2004).
- Santos, D. & de, C. et al. Forage dry mass accumulation and structural characteristics of Piatã grass in silvopastoral systems in the Brazilian savannah. Agric. Ecosyst. Environ.233, 16–24 (2016). ArticleGoogle Scholar
- Macedo, M. C. M. Crop and livestock integration: the state of the art and the near future. Rev. Bras. Zootec.38, 133–146 (2009). ArticleGoogle Scholar
- Tonucci, R. G., Nair, P. K. R., Nair, V. D., Garcia, R. & Bernardino, F. S. Soil carbon storage in silvopasture and related land-use systems in the Brazilian Cerrado. J. Environ. Qual.40, 833 (2011). ArticleCASPubMedGoogle Scholar
- de Freitas, E. C. S. et al. Litter fall and nutriente deposition on soil in an agrosilvopastoral system with eucalypt and acacia. Rev. Árvore37, 409–417 (2013). ArticleGoogle Scholar
- Montagnini, F. & Nair, P. K. R. Carbon sequestration: An underexploited environmental benefit of agroforestry systems. Agrofor. Syst.61–62, 281–295 (2004). Google Scholar
- Schoeneberger, M. M. Agroforestry: working trees for sequestering carbon on agricultural lands. Agrofor. Syst.75, 27–37 (2009). ArticleGoogle Scholar
- Lee, J., Ingalls, M., Erickson, J. D. & Wollenberg, E. Bridging organizations in agricultural carbon markets and poverty alleviation: An analysis of pro-Poor carbon market projects in East Africa. Glob. Environ. Change39, 98–107 (2016). ArticleGoogle Scholar
- Lal, R. Soil carbon sequestration impacts on global climate change and food security. Science304, 1623–1627 (2004). ArticleADSCASPubMedGoogle Scholar
- IPCC. 2006IPCC Guidelines for National Greenhouse Gas Inventories, Prepared by the National Greenhouse Gas Inventories Programme. (IGES, 2006).
- IPCC. Climate change2007: The Physical Science Basis. Contribution of Working Group I to the Fourth Assessment Report of the Intergovernmental Panel on Climate Change. (Cambridge University Press, 2007).
- Mosier, A. et al. Closing the global N2O budget: nitrous oxide emissions through the agricultural nitrogen cycle. Nutr. Cycl. Agroecosystems52, 225–248 (1998). ArticleCASGoogle Scholar
- Bouwman, A. F., Boumans, L. J. M. & Batjes, N. H. Emissions of N2O and NO from fertilized fields: Summary of available measurement data. Glob. Biogeochem. Cycles16, 6–1–6–13 (2002). Google Scholar
- Jones, C. D., Fraisse, C. W. & Ozores-Hampton, M. Quantification of greenhouse gas emissions from open field-grown Florida tomato production. Agric. Syst.113, 64–72 (2012). ArticleGoogle Scholar
- Esteves, S. N. et al. Estimativas da emissão de metano por bovinos criados em sistema de integração lavoura-pecuária em São Carlos, SP. 7 (Embrapa Pecuária Sudeste, 2010).
- Loetsch, F. & Haller, K. E. Forest inventory. (BLV Verlagsgesellschaft, 1973).
- Vital, B. R. Métodos de determinação da densidade da madeira. 21 (Sociedade de Investigações Florestais, 1984).
- Schumacher, F. X. & Hall, F. dos S. Logarithmic expression of timber-tree volume. J. Agric. Res.47, 719–734 (1933). Google Scholar
- Spurr, S. H. Forestry inventory. (Ronald Press, 1952).
- R Core Team. R: A Language and Environment for Statistical Computing. (R Foundation for Statistical Computing, 2013).
Acknowledgements
We acknowledge the financial support from Conselho Nacional de Desenvolvimento Científico e Tecnológico (CNPq, productivity grants), Coordenação de Aperfeiçoamento de Pessoal de Nível Superior (CAPES, Ph.D. scholarship, Grant No. BEX 10570/12-8) and Fundação de Amparo à Pesquisa do Estado de Minas Gerais (FAPEMIG, research funding). Thanks also to farmers Francisco de Freitas and Lino Roberto Ferreira for allowing us to work inside their properties. We also thank Lucas Arthur, Breno Loureiro, Gabriel Barros, Henrique Colares, Paulo Villanova, Bruno Schettini, Samuel José and Mateus Castro for laboratory and fieldwork. Dr. Phillip John Villani (The University of Melbourne, Australia) revised and corrected the English language used in this manuscript.
Author information
Authors and Affiliations
- Departamento de Engenharia Florestal, Universidade Federal de Viçosa, 36570-900, Viçosa, Minas Gerais, Brazil Carlos Moreira Miquelino Eleto Torres, Laércio Antônio Gonçalves Jacovine, Sílvio Nolasco de Olivera Neto & Carlos Pedro Boechat Soares
- Department of Agricultural and Biological Engineering, University of Florida, P.O. Box 110570, Gainesville, FL32611, USA Clyde William Fraisse
- TTG Brasil Investimentos Florestais Ltda, 18470-130, Itapeva, São Paulo, Brazil Fernando de Castro Neto
- Departamento de Fitotecnia, Universidade Federal de Viçosa, 36570-900, Viçosa, Minas Gerais, Brazil Lino Roberto Ferreira
- Departamento de Entomologia/BIOAGRO, Universidade Federal de Viçosa, 36570-900, Viçosa, Minas Gerais, Brazil José Cola Zanuncio
- Instituto de Ciências Agrárias, Universidade Federal de Minas Gerais, 39404-547, Montes Claros, Minas Gerais, Brazil Pedro Guilherme Lemes
- Carlos Moreira Miquelino Eleto Torres